Brain Damage Repair
Oussama KHERBOUCHE
Traumatic brain injury is a common cause of neurological disorders. It results in damage involving axons and other neighboring cells as well as surrounding tissue. The issue of these injuries lies in the fact that CNS regeneration is very limited. In contrast, the adult PNS regeneration potential is fostered. This led scientists to identify components responsable for this feature and find ways to tweak them in order to optimize post-traumatic CNS regeneration. Moreover, the discovery of spontaneous neurogenesis in specific brain regions has shifted the focus of research towards stimulating pre-existing neuron regeneration, as well as transplanting new precursor neuron, to help restore the brain’s lost functions.
Introduction
Neurology is one of the most underrated and complex fields of medical science. There are many unanswered enigmas and unturned stones, not only in pathophysiology of clinical presentations, but also in therapeutic approaches to diseases and pathologies. Scientists have acquired a sharp capacity to precisely localise neurologic lesions explaining possibly the underlying molecular mechanisms. Sadly, however, little is established about their therapies.
Nature didn’t make an easy task for human neurons to self regenerate and brain to self repair its damages, as it did for other organs such as muscles and bones, nor did it make the brain an easily accessible organ for manipulation. Moreover, neurologic disorders have severe dysfunctional consequences that could shackle the freedom of movement, cognition, sensing and many other functions. Head injuries are among major causes of these neurologic disorders, that we chose to review in our article.
Currently, each year in Algeria, 20,000 new head injuries are recorded. This figure is not exhaustive for it is not possible to provide reliable statistics in our country, due to the lack of serious studies on the phenomenon1. On the other hand, worldwide statistics estimate a number of 69 million individuals sustaining traumatic brain injuries (TBIs) each year2. They are caused largely by motor vehicle accidents, violence, combat injuries, fall or sport related injures which survivers are often left with disabilities ranging from long-term sensorimotor deficits, cognitive impairment to vegetative state3.
Accordingly, finding a cure to reverse the damages or at least to limit them is of an absolute necessity, to allow the neurologic injured people to live a functional and healthy life.
Therefore, in this article, we focus on the pathophysiology of TBI and on the different recovery processes of damaged tissues.
Consequences of CNS injury
Damages to the central nervous system (CNS) could involve any of its cells and tissues. Axons are the most at risk of injury, because they are so long that they are present in every corner of the nervous system, thus more exposed to injury.
The axotomy divides the axon in two: the distal and the proximal part.
On one hand, the distal part of cut axon responds to initial mechanical forces and mostly to secondary insults through the process of Wallerian degeneration. It is a process that begins with a reversible latent phase, then proceeds to an execution phase in which intra-axonal damage is irreversible and results in axon fragmentation 4, 5, 6.
It was long thought that the Wallerian degeneration was a passive process, following the lack of a nutritious supply after separation from the cell body. However, recent studies have shown the opposite. First, in mice mutants called Wlds (Wallerian degeneration slow), the distal axon persists 10 times longer than a normal separated distal axon. The Wlds mutation effect on degeneration postponing remains unclear, but suggesting the fact that degeneration can be slowed supports the idea that it is an active and not a passive process7. In addition, Nicotinamide mononucleotide adenylyltransferase (NMNAT), an enzyme that forms NAD+ and maintains axon health, which if reduced below a threshold level triggers the axon death pathway5. Furthermore, it was discovered that calpain, a calcium-dependent cysteine protease, becomes activated after axon injury, thus leading to axon degeneration 5.
In consequence, axon Wallerian degeneration is indeed an active process, that requires elevation of specific prodegenerative and reduction of protective molecules.
Identification of reversible versus irreversible stages of axon damage and dysfunction would provide important distinctions for repair capacity and relevant targets for intervention. For example, Calpastatin that inhibits calpain is used to prevent axon degeneration 8.
On the other hand, the proximal part of the cut axon suffers as well although it remains attached to the cell body. In some cases, the neuron itself dies by apoptosis, probably because axotomy isolates the neuronal cell body from its supply of target-derived trophic factors. When it survives, the cell body undergoes a phenomenon called “Chromatolytic reaction”: it swells , the RER (rough endoplasmic reticulum) fragments, the nucleus moves off center and many other metabolic changes occur. These changes are reversible though if regeneration happens 9.
Moreover, these neurons exhibit outgrowth from the proximal axon segment indicating regenerative capacity that could be targeted to improve repair 10 (Figure 1).
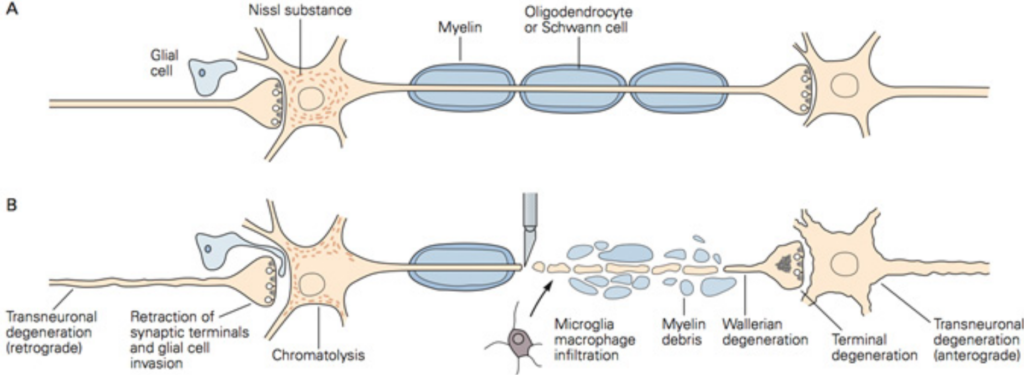
A. An intact neuron, axon wrapped by myelinating cells contacts a postsynaptic neuron.
B. Consequences of axotomy
Taken from: Eric R. K, John D. K, Sarah H. M, Steven A. S. Principles of Neural Science. In: Joshua R. S. Repairing the Damaged Brain. 6th ed. New York: McGraw Hill; 2021. p. 1237
Furthemore, axotomy sets in motion a cascade of responses in numerous types of neighboring cells, leading them to express different phenotypic and functional changes.
Indeed, this neuronal degeneration can propagate in both retro- and anterograde directions. Postsynaptic neurons undergo physiologic atrophy by disrupting inputs (as happens in denervated muscles). Presynaptic neurons are affected by what’s called « synaptic stripping », which is the withdrawal of synaptic terminals from the cell body of chromatolytic neurons and their replacement with processes of glial cells (Schwann cells in the periphery and microglia or astrocytes in the central nervous system), this prevents the afferent neuron from obtaining sufficient sustenance from its target cell 9.
Secondary damage to the central nervous system (CNS) is expected to cause myelin pathology that evolves throughout the post-injury time course by losing oligodendrocyte within and adjacent to the lesion region through damage by reactive oxygen species orginated from the injury and through caspase 3-mediated apoptosis 11, 12 with subsequent demyelination of viable axons. Myelin fragments associated molecules called myelin-associated inhibitors (MAIs) slowly diffuse throughout the CNS, inhibit axon regeneration13, 14, 15, 16 and stimulate microglial/macrophage activation 17. Thus, the myelin debris plays a role of axon regeneration inhibitor as well as proinflammatory.
Microglia and astrocytes are evenly not spared. Astrocytes normally serve multiple essential roles in the formation of functional excitatory synapses 18, 19. However, as a result of injury-induced circuit reorganization, as well as through inducing new membrane receptors for excitatory neurotransmitors in postsynpatic neurons in reponse to reduction or loss of excitatory input to these neurons following TBI, they can cause post-TBI epilepsy 20. Furthermore, reactive astrocytes generate scar tissue in the injured area 21, 22, 23.
Microglia become activated in response to injury, with diverse functional phenotypical changes that range from pro-inflammatory and phagocytic (M1-like) to immunosuppressive (M2-like) phenotypes that promote tissue repair, and growth stimulation 24, 25, 26, 27.
Molecules targeting nuclear hormone receptors that activate the M2-like phenotype, such as fenofibrate 28, 29, pioglitazone and rosiglitazone 30, 31, 32 have been proven to be effective in promoting regeneration. On the other hand, molecules attenuating M1-like phenotypes activation 33, 34, 35, 36, 37 were proven to be neuroprotective.
However, promoting M2-like microglias alone doesn’t lead to proper tissue repair. A long-term repair phase after a rapid pro-inflammatory response driven by M2-like macrophages has been demonstrated to result in fibrosis and other aberrant repair 38. Also, broad-spectrum anti-inflammatory therapies for TBI have translated poorly to the clinical outcome of human head injury 39, 40. Therefore, efficient tissue repair after TBI requires properly and timely coordinated transition between M1-like and M2-like microglias, that fine-tune the sequential inflammatory, proliferative and remodeling phases of active repair 41, 42. Thus, it is critical to boost, and diminish, the correct microglial and/or macrophage phenotypes at the right time in order to drive endogenous repair pathways following acute brain injury and avoid maladaptive responses.
CNS vs. PNS regeneration
The central nervous system (CNS) and the peripheral nervous system (PNS) differ essentially in their regeneration capacity. PNS has the possibility to recover after injury. Yet the distal axon segment doesn’t survive, but the proximal stump is able to grow sprouts and renew it. This renewal is controlled by chemotropic factors secreted by Schwann cells surrounding the distal stump and by adhesive molecules within it9. The Schwann cells and its connective tissue usually survive after axotomy. The regenerated axon reaches its target and forms a new synapse 43. Although this phenomenon is very impressive, it is not perfect: synaptic reconnection, when it happens, can be inappropriate. This results, for example in the motor system, in impaired fine movement but considerable strength recovery. In some cases, synaptic reconnection does not occur. The axon may fall short from its target and doesn’t reach it, as it may dissolve on its way 9 (Figure 2).
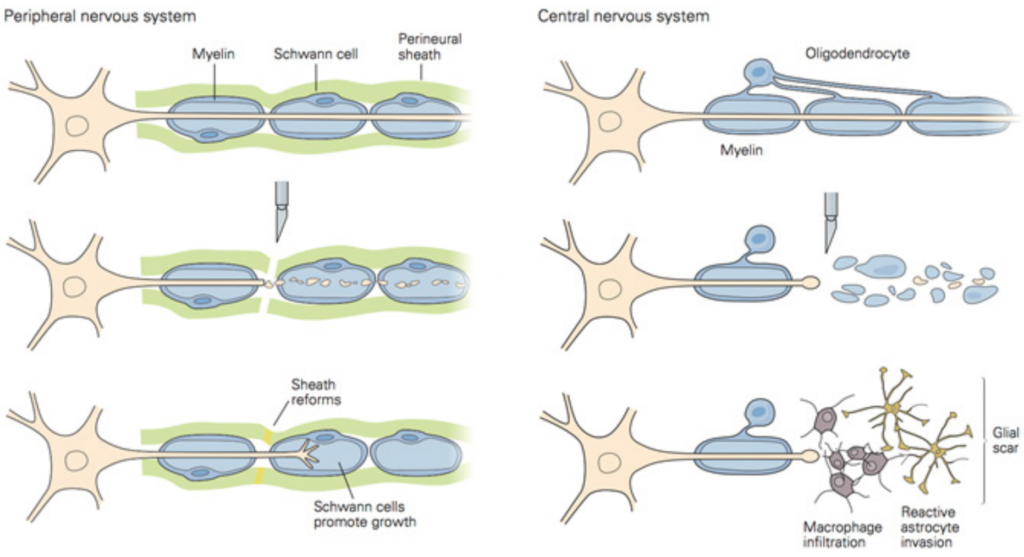
Taken from: Eric R. K, John D. K, Sarah H. M, Steven A. S. Principles of Neural Science. In: Joshua R. S. Repairing the Damaged Brain. 6th ed. New York: McGraw Hill; 2021. p. 1241
Conversely, the CNS regeneration after injury is very poor, if it happens at all. The proximal stumps of damaged axons can form short sprouts, but these soon stall and form swollen endings called “retraction bulbs” that fail to progress. Long-distance regeneration is rare 9. This has led to the pessimistic view that damage to the brain or spinal cord is irreversible, and that the only and unpromising therapeutic option is limited to rehabilition.
Interestingly, in mammals during developement, CNS transection can actually regenerate. Moreover, regeneration is vigorous in adult lower vertebrates such as fish and frogs 9. So the obvious question lighted up in the mind of neuroscientists: why did nature cause adult mammals to loose this substantial capacity to repair?
The possible answer could be linked to the brain cells’ important remodeling capacity during development that allows the newborn to adapt to its physiology and environement. But after remodling is settled, the brain does its best to keep it so it doesn’t loose its acquired functions 9, 44. For instance, during childhood, the brain reassigns cortical tissue to one eye after the other one is blinded for a long time. If this eye is recuperated, its function won’t come back. Fortunately, this doesn’t happen in case of a brief period of sight loss. That is why constant maintain of brain structure in the face of small perturbations in connectivity may be the price of losing regeneration capacitiy after injury 9. We could refer to that as « The pact with Satan », mammals’ CNS tissues have sold their restoration ability (soul) to acquire their precise wired system that sustains their superior intellectual faculties.
Besides, MAIs resorption after injury is faster in PNS than CNS. In the former, it is carried through by Schwann cells, which recrute macrophages from blood stream and produce growth factors, promoting axon regeneration. In contrast, The CNS relies on its remaining oligodendrocytes whose ability to resorb myelin is very reduced. In addition, the Blood Brain Barrier (BBB) precludes macrophage recruitment. Thus clearing debris is limited to resident microglias 45. This explains why Wallerian degeneration is slower in CNS than PNS.
Molecules regulating central neural regeneration
After discovering the different mechanisms of PNS and CNS regeneration, many scientists became motivated to try to manipulate different cells and environmental factors in order to optimize post-traumatic CNS regeneration. Albert Aguayo et al. found out in their experience in the 1980s that the central axons, when injured and transplanted in peripheral nerve environment, grew several centimers. Whereas, injured peripheral axons fared poorly when damaged and coupled with central nerve graft 46. These results suggest that the peripheral environement possesses growth-promoting factors normally absent in that of the CNS. Although these findings look very promising, central neurons regeneration in peripheral environment is unfortunately not as significant as natural peripheral regeneration, and therefore raise the following question: what are these factors, probably internal, required to promote a full regeneration of central neurons?
Several studies have succeeded in identifying components of peripheral nerves that are accountable for the neurite outgrowth. These include constituents of Schwann cell basal laminae such as Laminin, and cell adhesion molecules of the Ig superfamily. Moreover, distal nerve stumps produce neurotrophins and other trophic molecules. These components are important supplies for neurons to outgrow their axons, both in embryonic CNS and adult PNS. In adult CNS however, these constituents go astray and thus neurons lose their recovering ability 9. This motivated neuroscientists to experience supplementing the environment of damaged CNS with growth-promoting molecules. Indeed, they infused neurotrophins, peripheral extracellular matrix molecules such as laminine, trophic-factors-secreting trained cells or Schwann cells themselves into the target area 47, 48, yet the results remained disappointing: regeneration was limited and functional recovery was minimal. Consequently, it was hypothesized that a possible existence of inhibitory signaling pathways in the CNS blocked the growth promoting activity of cytokine factors.
As aforementioned, MAIs inhibit neurite outgrowth. Spinal injured rats treated with anti-myelin medication resulted in sprouting of spinal axon collaterals, suggesting that both central and peripheral environments have potential growth factors and confirming that myelin is a potent inhibitor of axon growth in the central nervous system 9. Additionally, this explains why myelinisation in the brain doesn’t occur directly after birth, allowing time for axon extension to complete. Numerous classes of MAIs found in central myelin have been identified: such as neurite outgrowth inhibitor (Nogo), myelin associated glycoprotein (MAG) and oligodendrocyte myelin glycoprotein (OMgp). Each of these proteins binds to common membrane receptors: Nogo receptors (NgR) and paired immunoglobulin-like receptors B (PirB). Mutant mice lacking PirB have enhanced regeneration of severed corticospinal axons 48, 49, 50, 51. Therapy targeting this common pathway is an interesting approach. In this context, a 66 hydrophobic amino-acid rich extracellular residue of NgR, which has the growth perturbing action of the receptor, hence named Nogo-66, has been targeted in many models by either antagonist peptides 13 or specific antibody production 52, which both resulted in nerve regeneration promotion and functional recovery in TBI models.
Another burden to axonal restoration in the CNS is the scar tissue generated by reactive astrocytes to the injured area 21, 22, 23. Indeed, scarring’s purpose is to limit the size of injury, reestablish BBB integrity and reduce inflammation, however it can hinder regrowth, which can be referred to as another « pact with Satan ». The ways the scar hinders regeneration are: First, through mechanical impediment of required space for axon growth. Second, through growth-inhbiting proteins produced by cells within the scar. Among these inhibitors are a class of chondroitin sulfate proteoglycans (CSPG), directly produced by reactive astrocytes that interact with tyrosine phosphatase receptors on axons. Chondroitinase, a treatement created on the purpose of decomposing CSPG and thus breaking down the glial scar 47, 48, 53, 54, 55.
Are the central neurons then condemned? Studies involving « conditioning lesions » refute this idea. The central branches of neurons of the dorsal root ganglia, that go into the spinal cord regenerate poorly, as it is in the CNS, contrary to the peripheric branches going from it, which regenerate well, as it is located in the PNS. However, if the peripheral branch is damaged a few days before the central branch, astonishingly the latter would regenerate well when the damage occurs. One explanation of that has been found to be the cyclic adenosine monophosphate (cAMP), which is present in high levels in embryonic and injured peripheral neurites and poorly in central neurites. Thereby, damaging the peripheric axon first leads to augmentation of cAMP in the neurone for a period in which it would help the central axon to regenerate if needed 9, 13. Likewise, drugs that increase cAMP or directly activate its targets are being developped with the intention of promoting central neurites recovery.
Moreover, an alternative to regeneration of cut axons have been found to be a rearrangement of neuronal connections. This favors positively but incompletely the functional recovery of target cells. For instance, several weeks after transection of the descending corticospinal pathway, new branches sprout from corticospinal axons rostral to the lesion and form synapses on spinal interneurons. These axons divert the injured ones by forming synapses with their former target cells 43, 56, 57, 58, 59. This phenomenom explains the latent plasticity of the nervous system. Therapeutic strategies are focusing on promoting this rewiring system as a support for axonal regeneration.
Central neurogenesis and precursor cells
It has long been thought that neurogenesis is complete by birth, thus therapeutic focus was on preserving the existing neurons. This dogma was however shattered by Jospeh Altman’s work in the 1960s, when he discovered that mature mammals’ brains have continous neurogenesis in regions called the subventricular zone (SVZ) surrounding the lateral ventricle, and in the subgranular zone (SGZ) of the dentate gyrus (DG) of the hippocampus 60, 61, 62, 63, 64, 65, 66 . The majority of the SVZ progeny are neuroblasts which undergo chain migration along the rostral migratory stream to the olfactory bulb, where they differentiate into olfactory interneurons 67. While, another sub-population of these cells migrate into cortical regions 68. Likewise, the newly generated cells of the DG differentiate into glial cells and a minority migrates laterally into the granule cell layer and becomes mature dentate granule neurons 69, 70, 71. Besides, damaged brain induces endogenous adult neurogenesis in non-neurogenic regions such as the striatum and cerebral cortex adjacent to the injury 72, 73, in a limited fashion, however.
Stem cells are the source of these new neurons in the adult as well as in the embryo. This discovery has reoriented the trajectory of research: First, into transplanting neuron precursors capable of division and differentation to restore the lost ones. Second, into stimulating this capability in pre-existing neurons in a vigorous manner to allow injured brain to regenerate.
Indeed, scientists have attempted for many years to experimentally transplant precursor neural cells. These trials were promising in animal models but more difficult to apply in humans. Obtaining a sufficient number of functional neurons seems to be problematic. Moreover, the grafted neurons are already too mature to differentiate adequately or to integrate effectively into functional circuits 74.
Progress was then made with neural transplantion, through discovering of new precusors such as neural stem (NS) cells. More often, these precursors differentiate into neurons corresponding more to the environment implanted in than to their origin site9. Suggesting that the environment plays a major role in differentation and that precursor cells plasticity is potent. However, such plasticity is limited. We need to set up differentiation of precursors along specific pathways in culture before grafting them.
More recently, new methods of precursor cells production have been developed. Embryonic stem (ES) cells derived from early blastocyst stage embryos potentially give rise to any cell of the body. These cells are directed toward a specific differention along specific pathways to generate transplantable cells. Subsequently, it became possible to generate then graft neurons corresponding appropriately to those lost in CNS injury or even in other brain disorders 75, 76.
Other transplantable cells are the induced pluripotent stem (iPS) cells generated from skin fibroblasts. The lack of political and ethical problems that are often met with ES cells procedures makes this method the preferred alternative. Another advantage of iPS cells is their easy acquisition from the patient’s own skin, avoiding moreover immunological incompatibilities 77, 78, 79, 80.
Moreover, as quoted earlier, some parts of our CNS also possess their own endogenous neuronal precursor cells (NPCs) capable of generating new functional neurons after injury. In fact, a study has found an increased number of cells expressing NS/NPCs markers in the perilesion cortex of the injured brain 81, strongly indicating the inherent attempts of the brain to repair and regenerate, and suggesting, that the specific areas of the brain, where full regeneration occurs, have some local permissive factors, that allows them to completely recover.
In consequence, this motivated the researchers to carry out animal experiments introducing specific growth factors drawn from progenitor cells grown in culture such as basic fibroblast growth factor , epidermal growth factor 82, 83 or S100β or vascular endothelial growth factor 84, 85, 86, which has led to improvement of injury-induced neurogenesis 87, 88. These results are very incentive and very tempting for human trials.
Moreover, oligodendrocytes are lost during brain injury as well. Although myelin-fragments are known to be potent inhibitors of axon growth, remyelinisation of axons is capital for their proper function. In reality, the adult brain and spinal cord can replace lost oligodendrocytes, but not in a sufficient manner capable of restoring function 56. Hence, studies have been focusing on transplanting oligodendrocyte precursors to regulate remyelinisation properly 74.
Conclusion
Understanding with minute detail the mechanisms of neuron regeneration in CNS and PNS, in embryos and adults, in humans as well as in animals, is capital to draw appropriate conclusions on factors necessary for optimal CNS regeneraton in adults after injury. Research on neural transplantation and stimulation of autoregeneration is making great progress. It promises to be the future cure to brain injuries seen almost daily in our hospitals.
In light of the fact that woodpeckers can withstand up to 10 times the maximum force collision between 2 american football players repeatedly, yet they suffer no head injury nor brain trauma 43. Neuroscientists have been inspired to look into the mechanisms of neuroprotection these birds use. Perhaps, some day they could extrapolate such knowledge on exposed humans such as athletes and prevent their brain from trauma damage instead of curing the consequent injury.
References
- Laouchedi M. Étude des circuits cérébraux de la conscience en IRM de diffusion par tractographie probabiliste: Application aux traumatismes crâniens sévère [dissertation]. Alger, Université des Sciences et de la Technologie Houari Boumediene. 2015.
- Dewan MC, Rattani A, Gupta S, Baticulon RE, Hung Y-C, Punchak M, et al. Estimating the global incidence of traumatic brain injury. Journal of Neurosurgery [Internet]. 2019 Apr [cited 2021 Sep 7];130(4):1080–97.
- Sun D. The potential of endogenous neurogenesis for brain repair and regeneration following traumatic brain injury. Neural Regen Res [Internet]. 2014 [cited 2021 Sep 7];9(7):688.
- Kelley BJ, Farkas O, Lifshitz J, Povlishock JT. Traumatic axonal injury in the perisomatic domain triggers ultrarapid secondary axotomy and Wallerian degeneration. Experimental Neurology [Internet]. 2006 Apr [cited 2021 Sep 7];198(2):350–60.
- Conforti L, Gilley J, Coleman MP. Wallerian degeneration: an emerging axon death pathway linking injury and disease. Nat Rev Neurosci [Internet]. 2014 Jun [cited 2021 Sep 7];15(6):394–409.
- Maxwell WL, Bartlett E, Morgan H. Wallerian Degeneration in the Optic Nerve Stretch-Injury Model of Traumatic Brain Injury: A Stereological Analysis. Journal of Neurotrauma [Internet]. 2015 Jun [cited 2021 Sep 7];32(11):780–90.
- Beirowski B, Berek L, Adalbert R, Wagner D, Grumme DS, Addicks K, et al. Quantitative and qualitative analysis of Wallerian degeneration using restricted axonal labelling in YFP-H mice. Journal of Neuroscience Methods [Internet]. 2004 Mar [cited 2021 Sep 7];134(1):23–35.
- Yang J, Weimer RM, Kallop D, Olsen O, Wu Z, Renier N, et al. Regulation of Axon Degeneration after Injury and in Development by the Endogenous Calpain Inhibitor Calpastatin. Neuron [Internet]. 2013 Dec [cited 2021 Sep 7];80(5):1175–89.
- Kandel ER, editor. Principles of neural science. 5th ed. New York: McGraw-Hill; 2013. 1709 p.
- Greer JE, McGinn MJ, Povlishock JT. Diffuse Traumatic Axonal Injury in the Mouse Induces Atrophy, c-Jun Activation, and Axonal Outgrowth in the Axotomized Neuronal Population. Journal of Neuroscience [Internet]. 2011 Mar 30 [cited 2021 Sep 7];31(13):5089–105.
- Lotocki G, Vaccari J de R, Alonso O, Molano JS, Nixon R, Dietrich WD, et al. Oligodendrocyte Vulnerability Following Traumatic Brain Injury in Rats: Effect of Moderate Hypothermia. Therapeutic Hypothermia and Temperature Management [Internet]. 2011 Mar [cited 2021 Sep 7];1(1):43–51.
- Flygt J, Djupsjö A, Lenne F, Marklund N. Myelin loss and oligodendrocyte pathology in white matter tracts following traumatic brain injury in the rat. Eur J Neurosci [Internet]. 2013 Jul [cited 2021 Sep 7];38(1):2153–65.
- Mukherjee N, Ghosh S. Myelin Associated Inhibitory Proteins as a Therapeutic Target for Healing of CNS Injury. ACS Chem Neurosci [Internet]. 2020 Jun 17 [cited 2021 Sep 7];11(12):1699–700.
- Israelsson C, Flygt J, Åstrand E, Kiwanuka O, Bengtsson H, Marklund N. Altered expression of myelin-associated inhibitors and their receptors after traumatic brain injury in the mouse. Restorative Neurology and Neuroscience [Internet]. 2014 [cited 2021 Sep 7];32(5):717–31.
- Geoffroy CG, Zheng B. Myelin-associated inhibitors in axonal growth after CNS injury. Current Opinion in Neurobiology [Internet]. 2014 Aug [cited 2021 Sep 7];27:31–8.
- Lang BT, Wang J, Filous AR, Au NPB, Ma CHE, Shen Y. Pleiotropic molecules in axon regeneration and neuroinflammation. Experimental Neurology [Internet]. 2014 Aug [cited 2021 Sep 7];258:17–23.
- Clarner T, Diederichs F, Berger K, Denecke B, Gan L, van der Valk P, et al. Myelin debris regulates inflammatory responses in an experimental demyelination animal model and multiple sclerosis lesions. Glia [Internet]. 2012 Oct [cited 2021 Sep 7];60(10):1468–80.
- Kucukdereli H, Allen NJ, Lee AT, Feng A, Ozlu MI, Conatser LM, et al. Control of excitatory CNS synaptogenesis by astrocyte-secreted proteins Hevin and SPARC. Proc Natl Acad Sci USA [Internet]. 2011 Aug 9 [cited 2021 Sep 7];108(32):E440–9.
- Christopherson KS, Ullian EM, Stokes CCA, Mullowney CE, Hell JW, Agah A, et al. Thrombospondins Are Astrocyte-Secreted Proteins that Promote CNS Synaptogenesis. Cell [Internet]. 2005 Feb [cited 2021 Sep 7];120(3):421–33.
- Hunt RF, Boychuk JA, Smith BN. Neural circuit mechanisms of post–traumatic epilepsy. Front Cell Neurosci [Internet]. 2013 [cited 2021 Sep 7];7.
- Burda JE, Sofroniew MV. Reactive Gliosis and the Multicellular Response to CNS Damage and Disease. Neuron [Internet]. 2014 Jan [cited 2021 Sep 7];81(2):229–48.
- Sofroniew MV. Astrogliosis. Cold Spring Harb Perspect Biol [Internet]. 2015 Feb [cited 2021 Sep 7];7(2):a020420.
- Sofroniew MV, Vinters HV. Astrocytes: biology and pathology. Acta Neuropathol [Internet]. 2010 Jan [cited 2021 Sep 7];119(1):7–35.
- Greenberg DA, Jin K. From angiogenesis to neuropathology. Nature [Internet]. 2005 Dec [cited 2021 Sep 7];438(7070):954–9.
- Colton CA. Heterogeneity of Microglial Activation in the Innate Immune Response in the Brain. J Neuroimmune Pharmacol [Internet]. 2009 Dec [cited 2021 Sep 7];4(4):399–418.
- Gordon S. Alternative activation of macrophages. Nat Rev Immunol [Internet]. 2003 Jan [cited 2021 Sep 7];3(1):23–35.
- Sica A, Mantovani A. Macrophage plasticity and polarization: in vivo veritas. J Clin Invest [Internet]. 2012 Mar 1 [cited 2021 Sep 7];122(3):787–95.
- Besson VC, Chen XR, Plotkine M, Marchand-Verrecchia C. Fenofibrate, a peroxisome proliferator-activated receptor α agonist, exerts neuroprotective effects in traumatic brain injury. Neuroscience Letters [Internet]. 2005 Nov [cited 2021 Sep 7];388(1):7–12.
- Chen XR, Besson VC, Palmier B, Garcia Y, Plotkine M, Marchand-Leroux C. Neurological Recovery-Promoting, Anti-Inflammatory, and Anti-Oxidative Effects Afforded by Fenofibrate, a PPAR Alpha Agonist, in Traumatic Brain Injury. Journal of Neurotrauma [Internet]. 2007 Jul [cited 2021 Sep 7];24(7):1119–31.
- Sauerbeck A, Gao J, Readnower R, Liu M, Pauly JR, Bing G, et al. Pioglitazone attenuates mitochondrial dysfunction, cognitive impairment, cortical tissue loss, and inflammation following traumatic brain injury. Experimental Neurology [Internet]. 2011 Jan [cited 2021 Sep 7];227(1):128–35.
- Thal SC, Heinemann M, Luh C, Pieter D, Werner C, Engelhard K. Pioglitazone Reduces Secondary Brain Damage after Experimental Brain Trauma by PPAR-γ-Independent Mechanisms. Journal of Neurotrauma [Internet]. 2011 Jun [cited 2021 Sep 7];28(6):983–93.
- Yi J-H, Park S-W, Brooks N, Lang BT, Vemuganti R. PPARγ agonist rosiglitazone is neuroprotective after traumatic brain injury via anti-inflammatory and anti-oxidative mechanisms. Brain Research [Internet]. 2008 Dec [cited 2021 Sep 7];1244:164–72.
- Bao WL, Williams AJ, Faden AI, Tortella FC. Selective mGluR5 receptor antagonist or agonist provides neuroprotection in a rat model of focal cerebral ischemia. Brain Research [Internet]. 2001 Dec [cited 2021 Sep 7];922(2):173–9.
- Byrnes KR, Stoica B, Riccio A, Pajoohesh-Ganji A, Loane DJ, Faden AI. Activation of metabotropic glutamate receptor 5 improves recovery after spinal cord injury in rodents. Ann Neurol [Internet]. 2009 Jul [cited 2021 Sep 7];66(1):63–74.
- Chen T, Zhang L, Qu Y, Huo K, Jiang X, Fei Z. The selective mGluR5 agonist CHPG protects against traumatic brain injury in vitro and in vivo via ERK and Akt pathway. International Journal of Molecular Medicine [Internet]. 2012 Apr [cited 2021 Sep 7];29(4):630–6.
- Loane DJ, Stoica BA, Byrnes KR, Jeong W, Faden AI. Activation of mGluR5 and Inhibition of NADPH Oxidase Improves Functional Recovery after Traumatic Brain Injury. Journal of Neurotrauma [Internet]. 2013 Mar [cited 2021 Sep 7];30(5):403–12.
- Wang J-W, Wang H-D, Cong Z-X, Zhang X-S, Zhou X-M, Zhang D-D. Activation of metabotropic glutamate receptor 5 reduces the secondary brain injury after traumatic brain injury in rats. Biochemical and Biophysical Research Communications [Internet]. 2013 Jan [cited 2021 Sep 7];430(3):1016–21.
- Hesse M, Modolell M, La Flamme AC, Schito M, Fuentes JM, Cheever AW, et al. Differential Regulation of Nitric Oxide Synthase-2 and Arginase-1 by Type 1/Type 2 Cytokines In Vivo: Granulomatous Pathology Is Shaped by the Pattern of l-Arginine Metabolism. J Immunol [Internet]. 2001 Dec 1 [cited 2021 Sep 7];167(11):6533–44.
- Kumar A, Loane DJ. Neuroinflammation after traumatic brain injury: Opportunities for therapeutic intervention. Brain, Behavior, and Immunity [Internet]. 2012 Nov [cited 2021 Sep 7];26(8):1191–201.
- Maas AIR, Roozenbeek B, Manley GT. Clinical trials in traumatic brain injury: Past experience and current developments. Neurotherapeutics [Internet]. 2010 Jan [cited 2021 Sep 7];7(1):115–26.
- Gurtner GC, Werner S, Barrandon Y, Longaker MT. Wound repair and regeneration. Nature [Internet]. 2008 May [cited 2021 Sep 7];453(7193):314–21.
- Novak ML, Koh TJ. Phenotypic Transitions of Macrophages Orchestrate Tissue Repair. The American Journal of Pathology [Internet]. 2013 Nov [cited 2021 Sep 7];183(5):1352–63.
- Bradbury EJ, McMahon SB. Spinal cord repair strategies: why do they work? Nat Rev Neurosci [Internet]. 2006 Aug [cited 2021 Sep 7];7(8):644–53.
- Ferretti P, Zhang F, O’Neill P. Changes in spinal cord regenerative ability through phylogenesis and development: Lessons to be learnt. Dev Dyn [Internet]. 2003 Feb [cited 2021 Sep 7];226(2):245–56.
- Vargas ME, Barres BA. Why Is Wallerian Degeneration in the CNS So Slow? Annu Rev Neurosci [Internet]. 2007 Jul [cited 2021 Sep 7];30(1):153–79.
- Aguayo AJ, David S, Bray GM. Influences of the glial environment on the elongation of axons after injury: transplantation studies in adult rodents. J Exp Biol. 1981 Dec;95:231–40.
- Bradbury EJ, Moon LDF, Popat RJ, King VR, Bennett GS, Patel PN, et al. Chondroitinase ABC promotes functional recovery after spinal cord injury. Nature [Internet]. 2002 Apr [cited 2021 Sep 7];416(6881):636–40.
- Liu BP, Cafferty WBJ, Budel SO, Strittmatter SM. Extracellular regulators of axonal growth in the adult central nervous system. Phil Trans R Soc B [Internet]. 2006 Sep 29 [cited 2021 Sep 7];361(1473):1593–610.
- Atwal JK, Pinkston-Gosse J, Syken J, Stawicki S, Wu Y, Shatz C, et al. PirB is a Functional Receptor for Myelin Inhibitors of Axonal Regeneration. Science [Internet]. 2008 Nov 7 [cited 2021 Sep 7];322(5903):967–70.
- Caroni P, Schwab ME. Antibody against myelin associated inhibitor of neurite growth neutralizes nonpermissive substrate properties of CNS white matter. Neuron [Internet]. 1988 Mar [cited 2021 Sep 7];1(1):85–96.
- Lee JK, Chan AF, Luu SM, Zhu Y, Ho C, Tessier-Lavigne M, et al. Reassessment of Corticospinal Tract Regeneration in Nogo-Deficient Mice. Journal of Neuroscience [Internet]. 2009 Jul 8 [cited 2021 Sep 7];29(27):8649–54.
- Lu X-M, Mao M, Xiao L, Yu Y, He M, Zhao G-Y, et al. Nucleic Acid Vaccine Targeting Nogo-66 Receptor and Paired Immunoglobulin-Like Receptor B as an Immunotherapy Strategy for Spinal Cord Injury in Rats. Neurotherapeutics [Internet]. 2019 Apr [cited 2021 Sep 7];16(2):381–93.
- Laabs T, Carulli D, Geller HM, Fawcett JW. Chondroitin sulfate proteoglycans in neural development and regeneration. Current Opinion in Neurobiology [Internet]. 2005 Feb [cited 2021 Sep 7];15(1):116–20.
- Galtrey CM, Fawcett JW. The role of chondroitin sulfate proteoglycans in regeneration and plasticity in the central nervous system. Brain Research Reviews [Internet]. 2007 Apr [cited 2021 Sep 7];54(1):1–18.
- Yiu G, He Z. Glial inhibition of CNS axon regeneration. Nat Rev Neurosci [Internet]. 2006 Aug [cited 2021 Sep 7];7(8):617–27.
- Bareyre FM. Neuronal repair and replacement in spinal cord injury. Journal of the Neurological Sciences [Internet]. 2008 Feb [cited 2021 Sep 7];265(1–2):63–72.
- Bareyre FM, Kerschensteiner M, Raineteau O, Mettenleiter TC, Weinmann O, Schwab ME. The injured spinal cord spontaneously forms a new intraspinal circuit in adult rats. Nat Neurosci [Internet]. 2004 Mar [cited 2021 Sep 7];7(3):269–77.
- Maier IC, Schwab ME. Sprouting, regeneration and circuit formation in the injured spinal cord: factors and activity. Phil Trans R Soc B [Internet]. 2006 Sep 29 [cited 2021 Sep 7];361(1473):1611–34.
- Schwegler G, Schwab M, Kapfhammer J. Increased collateral sprouting of primary afferents in the myelin-free spinal cord. J Neurosci [Internet]. 1995 Apr 1 [cited 2021 Sep 7];15(4):2756–67.
- Imayoshi I, Sakamoto M, Ohtsuka T, Takao K, Miyakawa T, Yamaguchi M, et al. Roles of continuous neurogenesis in the structural and functional integrity of the adult forebrain. Nat Neurosci [Internet]. 2008 Oct [cited 2021 Sep 7];11(10):1153–61.
- Zhao C, Deng W, Gage FH. Mechanisms and Functional Implications of Adult Neurogenesis. Cell [Internet]. 2008 Feb [cited 2021 Sep 7];132(4):645–60.
- Altman J, Das GD. Autoradiographic and histological evidence of postnatal hippocampal neurogenesis in rats. J Comp Neurol [Internet]. 1965 Jun [cited 2021 Sep 7];124(3):319–35.
- Lois C, Alvarez-Buylla A. Proliferating subventricular zone cells in the adult mammalian forebrain can differentiate into neurons and glia. Proceedings of the National Academy of Sciences [Internet]. 1993 Mar 1 [cited 2021 Sep 7];90(5):2074–7.
- Eriksson PS, Perfilieva E, Björk-Eriksson T, Alborn A-M, Nordborg C, Peterson DA, et al. Neurogenesis in the adult human hippocampus. Nat Med [Internet]. 1998 Nov [cited 2021 Sep 7];4(11):1313–7.
- Sanai N, Tramontin AD, Quiñones-Hinojosa A, Barbaro NM, Gupta N, Kunwar S, et al. Unique astrocyte ribbon in adult human brain contains neural stem cells but lacks chain migration. Nature [Internet]. 2004 Feb [cited 2021 Sep 7];427(6976):740–4.
- Walton NM, Sutter BM, Laywell ED, Levkoff LH, Kearns SM, Marshall GP, et al. Microglia instruct subventricular zone neurogenesis. Glia [Internet]. 2006 Dec [cited 2021 Sep 7];54(8):815–25.
- Doetsch F, Alvarez-Buylla A. Network of tangential pathways for neuronal migration in adult mammalian brain. Proceedings of the National Academy of Sciences [Internet]. 1996 Dec 10 [cited 2021 Sep 7];93(25):14895–900.
- Parent JM. The role of seizure-induced neurogenesis in epileptogenesis and brain repair. Epilepsy Research [Internet]. 2002 Jun [cited 2021 Sep 7];50(1–2):179–89.
- Lois C, Alvarez-Buylla A. Long-distance neuronal migration in the adult mammalian brain. Science [Internet]. 1994 May 20 [cited 2021 Sep 7];264(5162):1145–8.
- Kempermann G, Gage FH. Neurogenesis in the adult hippocampus. Novartis Found Symp. 2000;231:220–35; discussion 235-241, 302–6.
- van Praag H, Schinder AF, Christie BR, Toni N, Palmer TD, Gage FH. Functional neurogenesis in the adult hippocampus. Nature [Internet]. 2002 Feb [cited 2021 Sep 7];415(6875):1030–4.
- Arvidsson A, Collin T, Kirik D, Kokaia Z, Lindvall O. Neuronal replacement from endogenous precursors in the adult brain after stroke. Nat Med [Internet]. 2002 Sep [cited 2021 Sep 7];8(9):963–70.
- Bengzon J, Kokaia Z, Elmer E, Nanobashvili A, Kokaia M, Lindvall O. Apoptosis and proliferation of dentate gyrus neurons after single and intermittent limbic seizures. Proceedings of the National Academy of Sciences [Internet]. 1997 Sep 16 [cited 2021 Sep 7];94(19):10432–7.
- Thuret S, Moon LDF, Gage FH. Therapeutic interventions after spinal cord injury. Nat Rev Neurosci [Internet]. 2006 Aug 1 [cited 2021 Sep 7];7(8):628–43.
- Dunnett SB, Björklund A, Lindvall O. Cell therapy in Parkinson’s disease – stop or go? Nat Rev Neurosci [Internet]. 2001 May [cited 2021 Sep 7];2(5):365–9.
- Keirstead HS. Human Embryonic Stem Cell-Derived Oligodendrocyte Progenitor Cell Transplants Remyelinate and Restore Locomotion after Spinal Cord Injury. Journal of Neuroscience [Internet]. 2005 May 11 [cited 2021 Sep 7];25(19):4694–705.
- Dimos JT, Rodolfa KT, Niakan KK, Weisenthal LM, Mitsumoto H, Chung W, et al. Induced Pluripotent Stem Cells Generated from Patients with ALS Can Be Differentiated into Motor Neurons. Science [Internet]. 2008 Aug 29 [cited 2021 Sep 7];321(5893):1218–21.
- Takahashi K, Tanabe K, Ohnuki M, Narita M, Ichisaka T, Tomoda K, et al. Induction of Pluripotent Stem Cells from Adult Human Fibroblasts by Defined Factors. Cell [Internet]. 2007 Nov [cited 2021 Sep 7];131(5):861–72.
- Takahashi K, Yamanaka S. Induction of Pluripotent Stem Cells from Mouse Embryonic and Adult Fibroblast Cultures by Defined Factors. Cell [Internet]. 2006 Aug [cited 2021 Sep 7];126(4):663–76.
- Wernig M, Zhao J-P, Pruszak J, Hedlund E, Fu D, Soldner F, et al. Neurons derived from reprogrammed fibroblasts functionally integrate into the fetal brain and improve symptoms of rats with Parkinson’s disease. Proceedings of the National Academy of Sciences [Internet]. 2008 Apr 15 [cited 2021 Sep 7];105(15):5856–61.
- Zheng W, ZhuGe Q, Zhong M, Chen G, Shao B, Wang H, et al. Neurogenesis in Adult Human Brain after Traumatic Brain Injury. Journal of Neurotrauma [Internet]. 2013 Nov 15 [cited 2021 Sep 7];30(22):1872–80.
- Sun D, Bullock MR, McGinn MJ, Zhou Z, Altememi N, Hagood S, et al. Basic fibroblast growth factor-enhanced neurogenesis contributes to cognitive recovery in rats following traumatic brain injury. Experimental Neurology [Internet]. 2009 Mar [cited 2021 Sep 7];216(1):56–65.
- Sun D, Bullock MR, Altememi N, Zhou Z, Hagood S, Rolfe A, et al. The Effect of Epidermal Growth Factor in the Injured Brain after Trauma in Rats. Journal of Neurotrauma [Internet]. 2010 May [cited 2021 Sep 7];27(5):923–38.
- Kleindienst A, McGinn MJ, Harvey HB, Colello RJ, Hamm RJ, Bullock MR. Enhanced Hippocampal Neurogenesis by Intraventricular S100B Infusion Is Associated with Improved Cognitive Recovery after Traumatic Brain Injury. Journal of Neurotrauma [Internet]. 2005 Jun [cited 2021 Sep 7];22(6):645–55.
- Lee C, Agoston DV. Vascular Endothelial Growth Factor Is Involved in Mediating Increased De Novo Hippocampal Neurogenesis in Response to Traumatic Brain Injury. Journal of Neurotrauma [Internet]. 2010 Mar [cited 2021 Sep 7];27(3):541–53.
- Thau-Zuchman O, Shohami E, Alexandrovich AG, Leker RR. Vascular Endothelial Growth Factor Increases Neurogenesis after Traumatic Brain Injury. J Cereb Blood Flow Metab [Internet]. 2010 May [cited 2021 Sep 7];30(5):1008–16
- Magavi SS, Leavitt BR, Macklis JD. Induction of neurogenesis in the neocortex of adult mice. Nature [Internet]. 2000 Jun [cited 2021 Sep 7];405(6789):951–5.
- Shivraj Sohur U, Emsley JG, Mitchell BD, Macklis JD. Adult neurogenesis and cellular brain repair with neural progenitors, precursors and stem cells. Phil Trans R Soc B [Internet]. 2006 Sep 29 [cited 2021 Sep 7];361(1473):1477–97.
- Roberts NF. Bird Brains: Why Don’t Woodpeckers Get Concussions? [Internet]. Forbes. [cited 2021 Sep 7].